All published articles of this journal are available on ScienceDirect.
A Mini-review on the Effects of (Carbon) Nanoparticles and Oxidative Stress in Animals
Abstract
Background:
The carbon family nanoparticles are less reviewed for their impact on organisms associated with oxidative stress physiology.
Methods:
This review was carried out after collecting literature on the above topic from various sources, including PubMed and Google Scholar.
Results:
The carbon family nanoparticles have tissue-specific impacts on various organisms, which are evident at the molecular level.
Conclusion:
The carbon nanoparticles and molecules of its family need to be very judiciously released as waste to the environment as they may impart toxic effects on organisms.
1. INTRODUCTION
Studying oxidative stress and associated parameters has paramount importance for investigating the toxic effects or compatibility of a chemical agent. Biochemical analyses or molecular expression studies on oxidative stress and antioxidants are also used as biomarkers of stress to check the effects of environmental insults in animal models. Environmental changes, like exposure to chemicals, toxins, nanoparticles (NPs), metals, radiation, and change in pH, temperature, availability of food, salinity and climate change, may modulate the oxidative stress physiology of the organism [1, 2].
Oxidative stress can be regarded as a common indicator of toxicity, which causes tissue injury and alters the regulation of metabolic pathways. Oxidative stress is a result of an imbalance between generated oxidants and antioxidants present in our body, which leads to cell apoptosis or degenerative process. It is a consequence of the reduction in antioxidant levels due to the elevated generation of reactive oxygen species (ROS).
Lipids are the key constituents of every cell membrane. These are poised on a chain of hydrocarbons ending with groups of bonded oxygen and hydrogen having the general characteristics of partially not being water-soluble. Polyunsaturated fatty acids, which are abundant in cell membranes, are vulnerable to auto-oxidation. Free radicals, the chemically reactive molecules participating in a chain reaction, are likely to cause oxidative damage to the cell membrane [1, 2]. This process gains momentum by the presence of trace metals on exposure to O2. This process is well known as lipid peroxidation [1]. Lipid peroxides, upon decomposition, generate Malondialdehyde (MDA) and 4-Hydroxynonenal (HNE). Lipid peroxidation also causes damage to proteins and inhibits enzyme activity [2]. Therefore, lipid peroxidation (LPO) can be regarded as an oxidative stress indicator [1, 2].
Oxygen is a vital component of aerobic life. In the atmosphere, oxygen is usually unreactive, but metabolic activity and exposure to various environmental perturbations make it reactive, leading to the formation of free radicals. Oxygen, the elixir of life, turns a foe due to partial or incomplete reduction that leads to ROS generation in cells. As the free radicals have unpaired electrons present in open-shell configuration, their natural tendency to accept or donate electrons makes them chemically more reactive [3]. The ROS include superoxide anion (O˙ˉ2), hydroxyl radical (˙OH), hydroperoxyl radical (HO˙ˉ) and peroxy radicals, hydrogen peroxide (H2O2), lipid peroxides (ROOH), singlet oxygen (1O2) and hypochlorous acid (HOCl) [4]. Mitochondria are the primary site of ROS production. Electron escapes at coenzyme Q and complex I and reacts with molecular oxygen forming superoxide anion, a free radical that is further reduced to form other free radicals [5]. ROS production is a continuous process. The balanced ratio between ROS production and elimination leads to maintained cell health, whereas any substantial stress leads to overproduction of ROS [1-5].
Reactive oxygen species have an unavoidable pivotal role in several cellular processes. It has been known to serve as a cell-to-cell messenger and plays a major role in cell division, cell death, inflammation, and bactericidal activity of cells, like neutrophils. Phagocytic cells generate ROS to kill the engulfed microorganisms [6].
However, higher ROS production and accumulation damages all major macromolecules, like proteins, lipids, and nucleic acids, and results in deleterious modifications of those molecules [7]. Consequently, the antioxidant defense system gets activated to protect the cells from these harmful effects of ROS. Therefore, the study of antioxidant levels can be used to assess the oxidative (stress) state of animals [1, 2].
It is apparent that the oxidative insult coupled with the overproduction of ROS causes irregularities in biochemically regulated pathways [2]. Aerobic organisms have both enzymatic and non-enzymatic antioxidants, which help in reducing the deleterious effects of ROS [3]. Antioxidants reduce the free radicals by donating electrons without affecting themselves, and as a result, free radicals become less reactive. Their collective functions are meant to protect cells from oxidation caused by the produced ROS [3]. The enzymatic scavengers are superoxide dismutase (SOD), catalase (CAT), glutathione S-transferases (GST), glutathione reductase (GR), glutathione peroxidases (GPx), and glucose-6-phosphate dehydrogenase (G6PD). Whereas, ascorbic acid (AA) and reduced glutathione (GSH), carotenoids, alpha-tocopherol, and uric acid are considered potential non-enzymatic antioxidants [4].
Although the literature on “oxidative stress physiology” is plentifully available, that on its modulation by NPs in general and the carbon nanoparticles, namely by the reduced graphene oxide (rGO), in particular, is scant. Therefore, this review was intended to aggregate information on the effects of the toxicants and NPs in general and the rGO in particular on the oxidative stress in (aquatic) animals. Keywords, such as NPs, oxidative stress and reduced graphene oxide alone or in combination were searched in major scholar web engines, such as PubMed and Google Scholar, and articles based on the above keywords were selected in general and included in the review.
2. REDOX REGULATORY SYSTEM AGAINST OXIDATIVE STRESS
There are many enzymatic and non-enzymatic small redox regulatory molecules available to combat oxidative stress in organisms. They are enzymatic antioxidants or non-enzymatic small antioxidant molecules.
2.1. Superoxide Dismutase
The dismutation process of the superoxide (O2.-) radical to form molecular oxygen, O2 or H2O2, is catalysed by the SOD enzyme. In 1969, Joe M. Mc and Irwin Fridovich discovered SOD enzymes [5]. Depending upon the presence of metal cofactor (s), there are 3 major families of SOD present. They are the SOD enzymes containing copper, zinc, manganese, and nickel as co-factors. The mitochondrial SOD containing Ni as a cofactor is most commonly found in cells of prokaryotes and protists, whereas the other two, i.e. , Mg and Cu-Zn SODs, are found in eukaryotes [4].
2.2. Catalase
Catalase is the antioxidant enzyme named by Loew (1900) and crystallized by Sumner and Dounce (1937). Catalase (EC 1.11.1.6) is a tetramer located at the peroxisome. It is an important antioxidant belonging to the oxidoreductase class of enzymes, which catalyses the conversion of H2O2 molecules into water and oxygen [3, 4].
2H2O2 → 2H2O + O2
It plays a dual function depending upon the H2O2 concentration. At low concentrations of H2O2, it acts “peroxidatically” and at high concentrations, it decomposes the toxic H2O2 [8].
It also detoxifies other reactive species, like peroxynitrite, signifying an important role in cell defense against oxidative damage [9].
2.3. Glutathione-s-transferases
Glutathione s transferases (EC 2.5.1.18) are a family of iso-enzymes. They are dimeric and primarily located in the cytosol. They are best known for their detoxifying ability. The enzyme consists of a G site that binds to glutathione and a xenobiotic substrate binding region, which is relatively hydrophobic. This enzyme facilitates the conjugation of glutathione with exobiotic and endobiotic compounds, like carcinogens, environmental pollutants, NPs, drugs, or damaged cellular components, like lipid hydroperoxide and nucleotide hydroperoxide [10, 11], so they can be easily excreted through bile and urine protecting the cell from oxidative stress [12].
GSH + RX −−−−−−−−−→ GSX + RH
2.4. Ascorbic Acid
Ascorbic acid is a polar, organic molecule (sugar acid) (C6H8O6), produced by both plants and some animals (Fig. 1). It is a potential scavenger of ROS, such as O2˙‾, ˙OH, and HO˙, due to its high redox activity [7, 13]. Ascorbic acid can be readily oxidized by donating one or two electrons to free radicals and terminating the chain reaction of lipid peroxidation [14].
2.5. Glutathione (GSH)
In cells, GSH is the most commonly synthesized low-weighted thiol compound. Glutathione plays a crucial role in cell protection from oxidative damage and maintaining redox homeostasis. Glutathione was discovered by J.de Rey-Paihade in 1888 from yeast and animal tissue extracts.
There are mainly two forms of GSH, oxidized and reduced. The latter is more common in cells. The production house of GSH is the liver, in which the sulphur containing foods and relevant amino acids produce GSH. Mitochondria act as the major metabolic site for glutathione containing 10-15% of total cellular GSH.
2.6. Nanoparticles
The word ‘Nano’ is derived from the Greek word ‘Nanos’, which means dwarf or extremely small. Nanoparticles are a group of solid colloidal tiny particles with a dimension of roughly 1–100 nm. For ages, these have existed on the earth and formed from different natural phenomena, like volcanic eruptions, weathering of rocks, etc [15]. Incidentally, NPs are rejected by the environment in the form of diesel exhaust, coal combustion, welding fumes, etc.
The NPs are small in size and have different physical and chemical properties, and also have a larger surface area. These properties make NPs of greater interest than their counter bulk parts.
2.6.1. Different Types of NPs
Nanoparticles are broadly divided into various categories based on physical and chemical characteristics. Mostly used NPs are classified as metallic, ceramics, polymeric, lipid-based, and carbon-based NPs. Among all NPs, carbon NPs are the most promising engineered material. Due to their ability to conduct electricity, heat, and their extreme flexibility, carbon NPs are widely used for many commercial and industrial purposes. Carbon NPs are the second-highest nanoparticles used among all the available NPs in the global market [16]. The members of carbon NPs are fullerenes, carbon nanotubes, nanowires, etc.; the new members added to this family are graphene, graphene oxide, and reduced graphene oxide (Fig. 2).
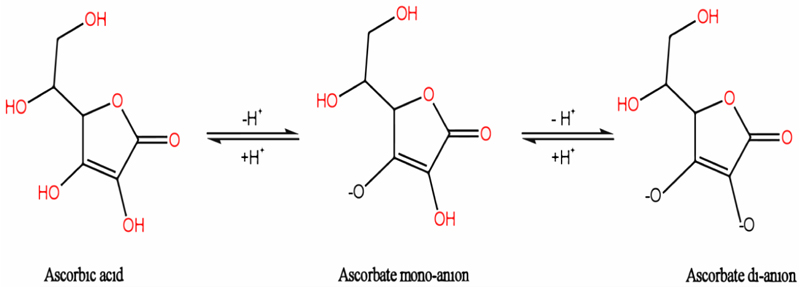
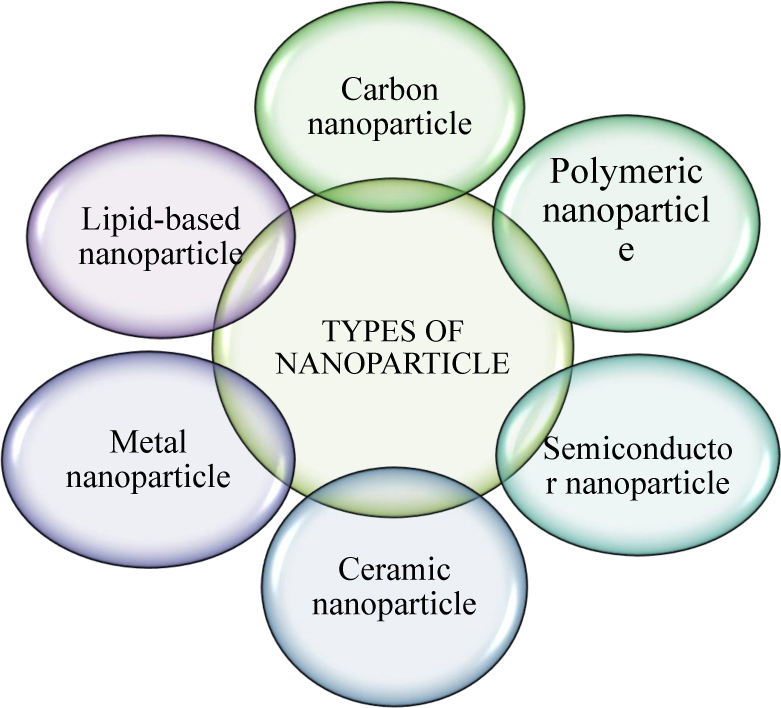
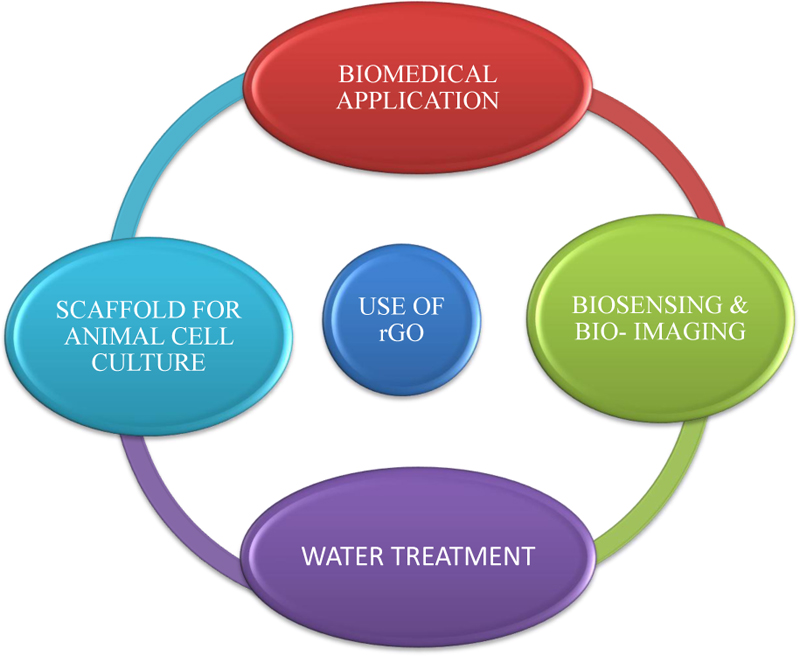
2.6.2. The Carbon and its Derived NPs
Carbon, the most abundant element on earth, shows catenation. Thanks to this property, more numbers of allotropes are formed. Reduced graphene oxide belongs to the graphene family of nanomaterial (GFNs), and graphene oxide is reduced to make rGO. Chemical, thermal and photo-thermal methods are used to obtain reduced graphene structures.
The carbon/oxygen ratio is higher in rGO than in GO. The rGO contains oxygen residues and other oxygen-containing functional groups on its basal planes, along with defects or holes. rGO can be used in energy storage, composite materials, semiconductors, biomedical applications, etc [17].
The increased usage of NPs has resulted in their release into the water bodies, causing unknown hazards to humans and aquatic life. In recent years, the potential of NPs to react with the biological systems has been identified, such as enhanced production of ROS, which leads to OS causing severe damage to cellular macromolecules (Fig. 3).
Nowadays, nanomaterials are often used for coatings on metals, sewage treatment, in military appliances, and for making cosmetics. Reduced graphene oxide can be used for preparing batteries, super-capacitors, electric materials, and biomedical instruments. Nanoparticles can be used for bio-catalysis to act as an ideal matrix for protein or enzyme immobilization.
2.7. Effects of NPs on Oxidative Stress in General
Nowadays, various industries like agricultural, pharmaceutical, plastic, etc., involve the large-scale production of NPs. As it is evident that the industrial waste ends up in water bodies, the NPs released into the aquatic environment may cause deleterious effects on aquatic lives. Due to their tiny size and shape, they easily get dissolved in water bodies; as a consequence, aquatic organisms may face subsequent effects, such as mechanical and physiological damage.
NP toxicity is a complicated mechanism that affects cellular metabolism by affecting the biochemical pathways. Concomitantly, the NPs stimulate ROS overproduction and cause an imbalance in antioxidant levels, leading to oxidative stress [18]. Thus, changes in antioxidant levels and monitoring the oxidative state of aquatic animals can be used as an indicator for a variety of contaminants and toxicants. Recently, researchers have been interested in knowing the effects as well as finding the remedial measures of nanoparticle toxicity. Besides the above and external agents, such as NPs, many cellular physiologies have also been found to be related to ROS generation and oxidative stress. For example, autophagy is a process in living organisms closely related to ROS utilization. It is one of the main routes to eliminate damaged components in cells in response to oxidative stresses. ROS may initially oxidize several enzymes, including autophagy proteins, to inhibit the entire process. ROS then trigger signalling pathways to activate autophagy to form a negative feedback loop to suppress ROS [16, 18]. However, to know the effects of nanoparticles’ toxicity on aquatic life, the antioxidant levels and oxidative stress parameters of model organisms have been examined (Fig. 4).
2.8. Effects of Nanomaterials on Aquatic Organisms
In the last decade, nanomaterials have become an unavoidable part of the present-day lifestyle. Due to their extensive use and intentional or unintentional release of nano-sized by-products into the aquatic environment, the environment, as well as its organisms, can be affected. The biological response to nanoparticle (NP) exposure can be measured by several parameters, such as the presence or absence of organisms, growth, morphological changes, biochemical changes, oxidative state, or generation of ROS. The sequential order of responses to anthropogenic and environmental stress within a biological system is as follows [19].
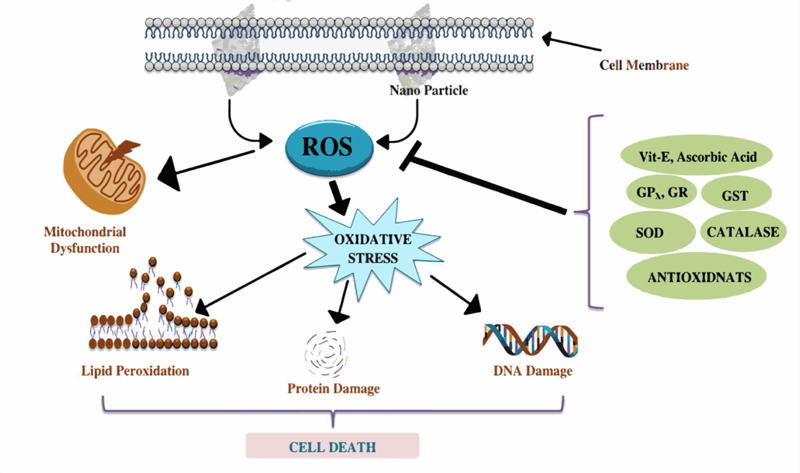
Molecular → Organelle → Cellular → Tissue Level → Systemic → Organism → Population → Community → Ecosystem
Thus, research on molecular and biochemical parameters has been triggered, as it reflects the presence and potency of toxicants. Although NPs are useful in various sectors, their excess use entails a risk to both organisms as well as the environment. It has been found that NP induces an increased expression of ROS depending on the exposure time, concentration, and size of NPs.
Nanoparticles are released into all the ecosystems on the earth. Nanoparticles can enter an aquatic environment from (1) waste-water treatment plants effluents, (2) direct use, and (3) deposition from their compartments. Different factors, like unique properties, half-life, and the response of organisms to NPs determine the effect of NPs [20].
Colloidal particles, like inorganic and organic matter, originating from natural as well as anthropogenic sources react with the NPs, resulting in different transformations owing to changes in their bioavailability [21]. Low hydrophilicity and instability of the aqueous suspensions limit the accessibility of most of the NPs for aquatic organisms; however, the NPs penetrate the organisms through the skin via airborne route or with water and food [22]. The study of the effects of NPs on marine invertebrates showed the occurrence of immunomodulation and oxidative stress, leading to the death of tissues [23]. An in vitro study on M. galloprovincialis showed that the carbon NPs and metal oxides jeopardized various biochemical processes and also induced apoptosis [24].
Nanomaterials act in a concentration-dependent manner, as at low concentration, they promote growth, and at higher concentration, they cause deleterious effects on aquatic organisms. With the increased concentrations of NPs, like Ag and ZnO, the microbial population decreases, and the activity of ammonia-oxidizing bacteria is also inhibited by AgNPs [25, 26]. On exposure to cerium oxide, the breakdown of DNA strands occurred in Daphnia magna; contrary to this, no effect was seen in the growth and mortality of Chironomous riparius [27]. CuNPs induced gill damage and decreased Na+-K+ ATPase activity in a dose-wise manner (0.25–1.5 mg/l), with a 48-hour LC50 value of 1.5 mg/l in Zebrafish [28]. CuO NPs also have a high ecotoxic effect on neotropical fish species, like Ceriodaphnia silvestrii and Hyphessobrycon eques, leading to the production of ROS [29]. The engineered NPs, due to the inherent physical and chemical properties and the presence of co-pollutants, show a disruptive genotoxic effect [30]. TiO2 causes oxidative damage to algal cells by making aggregation and also causes ocean acidification. Due to ocean acidification and long-term exposure to zinc oxide, enhancement in the activity of mussel enzymes, such as catalase, occurred, and also damage to the lipid bilayer was seen [31].
2.8.1. Negative Effects of Nanomaterials
At high concentrations, nanomaterials can negatively affect the growth and anatomy of living organisms. Bacteria are less affected by NPs due to their ability to develop a defense system. Nanoparticles can cause damage to DNA and protein [18]. They can cause oxidative stress by disrupting the integrity of membranes and entering into the cells.
It has been reported that NPs affect the mortality, reproduction, heart rate, feeding rate, and swimming velocity in Daphnia magna, a planktonic crustacean [32]. Exposure to NPs at low trophic levels may cause harm at higher trophic levels to the food web due to bio-accumulation [18]. In 2008, Mouchet et al. also reported such accumulation of NPs in the gut of tadpoles [33]. The adsorption of carbon NPs, like fullerene [34] and diamond NPs [35], onto aquatic organisms, such as algae [36], has been reported. Such adsorption of NPs on the organism is called ‘biological surface coating’, which may adversely affect food uptake by herbivores.
A study on the effects of heavy metals has revealed that polychaetes exposed to copper showed induction in SOD and GST genes [37] to combat the OS. Another study related to the effect of NPs on largemouth Bass fish showed a higher rate of LPx in the brain of the fish [34], and a study on Pimephale promelas, a fathead minnow, treated with tetrahydrofuran (THF) prepared fullerene (nC60), showed 100% mortality.
Zebrafishes are the most used model organism for toxicity studies because of their rapid development and small size. Studies [38, 39] show that long-term exposure to NPs can cause oxidative stress in the gills and liver of Danio rerio fish. A study on the dose-dependent effect of NP on rainbow trout fish resulted in increasing levels of glutathione up to 28% and 18% in the gill and liver, respectively. However, in some cases, it also showed mortality [40]. Zhang et al. [41] reported that the co-addition of TiO2 NPs enhanced the cadmium accumulation in fish, and this higher accumulation factor of TiO2 directly led to an increased intake of the heavy metal. Moreover, an iron-rich diet in African Catfish (Clarias gariepinus) was found to induce LPx in the heart and liver of the animal [42]. Studies on several different snails, such as Eobania vericulata, Helix aspersa, and Gibbula umbilicalis, have also reported increased GST activity with increasing xenobiotic concentration in hepatopancreas tissue [31]. Different studies have revealed that snails exposed to ZnO NPs also induce LPx rise with a significant decline of antioxidant activities, like glutathione-S-transferases in the hepatopancreas, as a result of oxidative stress.
Direct or indirect exposure to NPs can cause health risks to humans. Inhalation of water aerosols or consumption of contaminated drinking water containing a trace amount of NPs is a possible way of their direct contact through the skin. The consumption of plants or animals that have grown in such contaminated water is an example of indirect way [18].
In Fish: Young salmons were treated with Ag NPs commercial suspensions and those prepared with AgNO3 reduced with NaBH4. Metal NPs are more toxic than dissolved ones; it has been proved in the case of Zebrafish using Cu NPs and LC-50. The activities of ZnO-NPs were observed on in vitro cultures of hepatocytes or hepatic cells obtained from human and fish models. Fish behaviour was found to be altered in response to light stimuli. The effects of TiO2-NPs and C-NPs were analysed in the case of trout liver cells [43]. Toxicity evaluation of CuO bulk and NPs in Tilapia was also studied. Both Cu2+ and Zn2+ ions are toxic and complicate the interpretation of CuO and ZnO NP toxicity.
In Crustaceans: The toxic effects of NPs have also been studied on crustacean species, such as Daphnia or T. platyurus. In Daphnia, AgNO3 mainly affected reproduction and growth. Daphnia exposed to TiO2-NPs-contaminated algae showed an accumulation of Ti inside the digestive tract [44].
In Echinoderms and Molluscs: Echinoderms and molluscs represent good animal models to study the toxic roles of NPs. The effects of reactions of metallic oxide-NPs, like SnO2-NPs, CeO2-NPs, and Fe3O4-NPs, were tested on the defense mechanism of sea urchin. NPs were accumulated in coelomocytes, which are present in cells of the immune system of sea urchin [45]. In bivalve molluscs, immune functions are targeted by NPs. The effects of NPs on the defense mechanism were experimentally studied in Mussel Mytilus. Various NPs affect several parameters, like lysosome activities, phagocytic activity, generation of free radicals, or increase in apoptosis. In Mytilus, TiO2-NPs could paste on the gills, join directly with the digestive glands and penetrate cells with consequent lysosomal perturbation and microbial peptides [46].
2.8.2. Positive Effects of Nanomaterials (Antioxidant, Antifungal, Antibacterial Properties)
The impact of nanotechnology is described in terms of its medical, ethical, psychological, legal, and environmental applications to areas, such as engineering, bioscience, chemical science, computing, material science, and communications.
Nanomaterials are very promising frontiers in the production of improved antioxidants. Some nanomaterials, including organic (melanin, lignin) metal-oxide (gold, platinum) based NPs, exhibit intrinsic redox activity, which is often associated with radical trapping or with SOD and catalase-like activities. Antioxidant study methods confirmed that silver (Ag) NPs have more antioxidant activity as compared to vit-C.
Phenols, diarylamines, and ascorbate have weak N-H or O-H bonds and act as formal hydrogen-atom donors. NPs advance the technologies of bio-imaging. The two main types of such NPs are luminescent nanoprobes for OI and magnetic NPs for MRI.
NPs are increasingly used as an alternative to antibiotics. The use of NPs for coating antibacterial compounds for medicinal use in order to prevent bacterial infections, for wound healing, in bacterial detection systems, to generate microbial diagnostics, and to produce antibacterial vaccines, is common.
Silver is the most precious metal used in the preparation of NPs because of its great antimicrobial activities. Engineered NPs can be used as fungicides. MgO NPs have antifungal activity against P.nicotianae. AgNPs may kill fungal spores by destroying the membrane integrity. Cu NPs demonstrate significant antifungal activity.
Graphene quantum dots (GQD) have been widely used in pharmacological industries for making various medicines due to their antioxidant activities [47]. Carbon nanomaterial’s possible role in cell proliferation has been reported in recent studies [48], since carbon is a potential source for growth.
Nanomaterials like AgNPs and titanium dioxide (TiO2), copper-containing compounds, such as CuSO4 and Cu(OH)2, zinc oxide (ZnO), iron NPs, fullerene derivatives, and carbon nanotubes have antimicrobial properties [49, 50]. Gold NPs are also known to have antibacterial properties. They can collapse cell membrane and inhibit ATPase activity [51]
Graphene oxide and rGO have toxic effects on most bacteria. Sharp edges of GO and rGO can induce membrane stress, leading to the disintegration of the membrane and RNA leakage [52]. Graphene-Fe3O4 composite aggregates the bacterial proteins and acts as a bactericide. Graphene oxide nanosheets have antifungal activities, as their sharp edges cause damage to the plasma membrane of pathogenic cells. Graphene oxide-Ag nanocomposites also act as antifungal agents. ZnO-GO, TiO2-GO, Ti-GO-Ag, and CuO-rGO composites have been explored to have antibacterial properties [53]. A study on Escherichia coli has shown that silver NPs have excellent antibacterial properties [54].
According to a study, rGO shows an antibacterial effect on E.coli at a relatively high concentration, but at a low concentration, it shows a reverse effect that facilitates the growth of bacteria [48]. The importance of GO and rGO in the nano-industry draws special attention.
3. EFFECTS OF CARBON NP DERIVATIVES ON ORGANISMS
A graphene-based nanomaterial on interacting with different natural organic materials in an aquatic environment can cause many body deformities in various organisms. GO-induced PLHC-1 cells showed a notable decrease in the membrane potential of mitochondria (MMP) as well as an increase in ROS levels [55]. GO nanosheets on direct contact with cell membranes cause stress. As a result of this damage to the cell membrane, the lipid bilayer gets ruptured, which leads to cell death. Excessive generation of ROS engendered protein carbonylation and DNA modification on exposure to GO at concentrations of 1-100 mg/l, ceasing the development of zebrafish embryos [56]. Oxidative stress and enzyme activity got enhanced after the injection of GO along with an increase in HSP70 level in Acheta domesticus, a native grasshopper in South-Western Asia [57]. GO was also able to increase oxidative stress by producing ROS in the cell and mitochondria of Anabas testudineus, as evidenced by elevated lipid peroxides, alteration in enzyme activity, and changes in the protein level [58]. In Caenorhabditis elegans, protein-protein interaction was greatly inhibited due to the inoculation of GO [59]. In Crassostrea virginica, a filter-feeding marine bivalve, exposure to agglomerates impregnated with GO harmed its physiology. A tissue-specific study on this species showed elevated levels of lipid peroxidation and subsequent changes in antioxidant activities denominating oxidative stress [60]. In a similar study on short-term exposure of 72 hr, an elevated level of lipid peroxidation was seen in a dose-dependent manner with GO concentrations of 1 and 10 mg/l, whereas prolonged or long-term exposure to GO showed no such significant changes in the antioxidant level. Regular metabolism got hampered by coexposure of GO to trace elements of certain concentration (GO 1.0 mg/l) in addition to trace elements, like Cd 1.0 mg/l and Zn 1.0 mg/l, in Palaemon pandaliformis [61]. On subjection to GO and its derivatives at different concentrations in bluegill sunfish, a significant increase in the level of oxidative stress indicators, like lipid peroxidation and CAT, was noticed [62].
Oxygenic functional groups present on the surface act as the primer, which enhances the adsorption capacity of graphene materials [63-65]. GO having more oxygenic groups than other GFNs are more susceptible to the interaction with heavy metals in aquatic environments [66]. A study on Cu toxicity to GFNs revealed the fact that graphene materials had eased Cu accumulation by scaling up the oxidative stress in Daphnia magna, whereas the mortality got decreased with an increment in Cu concentration up to 50µgL-1 [67].
Due to the hydrophilic nature of GO, it becomes suspended in the sediments of the aquatic environment and serves as food for a myriad of aquatic organisms. The antioxidant defense capacity study in clams indicated that at a higher concentration of GO, the catalase activity became decreased owing to enzyme inhibition and potential induction of peroxy radical [68]. In vivo toxicity studies of GO and manganese ion contaminated GO showed an increase in catalase activity conforming to the intensification of oxidative stress after the subjection of GO [57].
Graphene is a single-atom-thick, carbon-based nanoparticle in which sp2 bonded carbon atoms are densely packed hexagonally [69]. Internalization of graphene generally causes damage to the plasma membrane and induces cytotoxicity [70]. At the site of interaction between the graphene and the cell membrane, membrane invagination and some disruption occur [71]. Graphene in the lowest concentration can cause genotoxicity, whereas NPs of SiO2, ZnO, TiO2, Sn, and carbon nanotubes induce DNA damage only at higher concentrations [72].
The biocompatibility of graphene and its derivatives varies in terms of their shape, size, and surface area. They have attracted great interest in many sectors due to their electronic, magnetic, and mechanical properties [69].
Graphene oxide, a derivative of graphene, has hydrophilic properties, smoother edges, and high oxygen content, and it can cause cytotoxicity [73] in some organisms. In an aquatic environment, the negative charge of GO makes it a great adsorbent of positively charged pollutants. Cellular internalization of GO causes intoxication and mitochondrial dysfunction by decreasing the mitochondrial membrane potential and imbalance in mitochondrial calcium homeostasis, and it leads to the overproduction of ROS. Graphene oxide might act as an electron donor that increases the supply of electrons to the Electron Transport Chain (ETC), and as a result, it generates ROS, mainly including hydroxyl radicals, as a by-product of mitochondrial respiration [71, 41].
In a study on eastern oyster (Crassostrea virginica), graphene oxide-induced LPx was reported to induce a decline in protein levels, and no significant changes in GST activity were observed. Graphene oxide toxicity test on Diopatra neapolitana, a polychaete, showed an adverse impact on growth and regenerative capacity [74]. HepG2 cells after 48 hours of exposure to GO showed an increase in ROS levels and mitochondrial damage [75]. It has been reported that GO treatment significantly increases ROS levels, decreases antioxidant activity, and induces LPx in HeLa cells [41]. Administration of GO has shown to cause an increase in LPx and ROS levels and lead to decreased action of antioxidants like SOD, CAT, and GPx in H9C2 cells [73].
Because of their chemical natures, graphene-based nanoparticles (GPN) are considered one of the most important types of NPs used in various fields. Graphene (GN) and graphene derivatives have various toxic effects on the aquatic environment. GN is the new allotrope of ‘C’, and is defined as a single layer of mono-crystalline graphite with carbon atoms closely packed in a 2-D honeycomb lattice, resulting in a large surface area on either side of the planar axis [76]. Experimental studies indicate that- GPN accumulates in the body of living creatures and causes various threats to the developing foetuses [77].
The production and the outcome of GP-based materials throughout the world indicate the release of GPN into the environment, including one layer GP, few-layer GP (2-10 layers), GP nanosheets, GP ribbons, GP oxide, rGO, graphene oxide (GO), GP quantum dots (GQD), and reduced GQD (rGQD). The discovery of GO was first made during the harsh oxidative treatment of graphite in 1859. The effects of GO on zebrafish, Danio rerio, were studied. Structural analysis revealed injuries to gill cells that were in an early apoptotic stage. There was also observed ROS generation in gills, necrosis, lesions in liver tissues, clubbed tips, lamellar fusion, etc [38].
Topminnow fish hepatoma cell line PLHC-1 cells were exposed to monolayer GO and carboxyl graphene (CXYG) suspensions along with an aryl hydrocarbon receptor. The results showed that the pre and co-exposure of cells to GO and CXYG nanoplatelets induced cytochrome P4501A expression, suggesting that graphene nanoplatelets increased the effective concentration of AhR agonists by facilitating passive diffusion into cells by impairing plasma membrane or displacing them over the plasma membrane through Trojan-horse like mechanisms.
Zebrafish embryos were exposed to GQD (2.3-6.4nm) 0-dimensional carbon-based materials at 12.5, 25, 50, 100 and 200 μg/ml concentration, 4-120 hpf in E3 medium containing 10-15% methylene blue. The data indicate that GQD was mainly incorporated in the myocardial cytoplasm, and the heartbeats were reduced only at a higher concentration of GQD from 50-120 hpf [78].
The versatile role of GO-derived NPs, as indicated above, leads to a common consensus that their special structure in each form plays a specific role in living organisms. Therefore, a detailed account of the structural aspects of the GO-derived NPs, especially that of rGO, needs to be linked with its specific role.
4. STRUCTURE OF THE rGO
The nanoparticle rGO belongs to the graphene family of nanomaterials. Graphene family of nanomaterials, due to their physicochemical structure, are in skyrocketing demand in many industrial applications and particularly in the biological field. Reduced graphene oxide is a reduced form of GO. It is produced by reducing the oxygen content of GO. The reduction can be accomplished with various methods based on chemical, thermal or electrochemical techniques. Amongst these techniques, some can manufacture high-quality rGO, which is comparable to pristine graphene. Pristine graphene is pure graphene in its unoxidized form. Chemically reduced GO creates poor quality rGO concerning the surface area and electrical conductibility. By the process of thermal reduction, GO can be reduced to rGO having a large surface area (Fig. 5). This results in a reduction in the mass of the GO. This creates imperfections and holes in the structure, and it affects the mechanical strength. It has been shown that very high-quality rGO is produced by using the electrochemical method [79, 80].
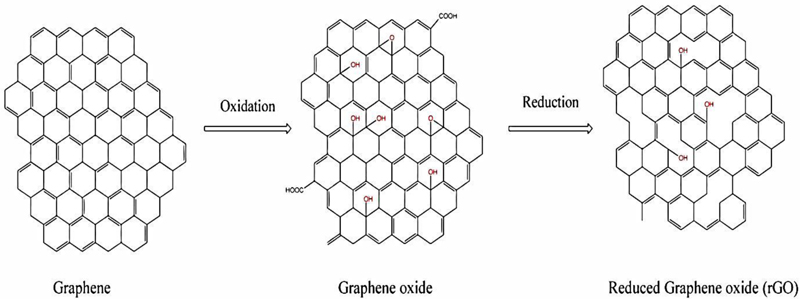
Reduced graphene oxide was characterized by energy-dispersive X-ray spectroscopy (EDX) and Raman Spectrum, which revealed remaining oxygen contents, defects, and the stretching of sp2 atoms, after the reduction reaction. In FTIR studies, the stretching vibration of O–H, C=C, C=O, and O–H deformation implied the removal of original functional groups in rGO [81].
Due to the removal of oxygen functional groups, rGO creates aggregates rather than dispersing in water [17]. The residual oxygen groups make rGO a faulty material. It becomes extremely useful for high conductivity as well as for the production of polymer composite materials because of the defects generated after reduction [79]. Removal of oxygen-containing bonds results in partial restoration of π bonds among carbon atoms. Reduced GO is negatively charged due to the residual oxygen functional groups [81, 82].
Applications of rGO have been fueled by its specific characteristics, such as high specific surface area, high electron mobility and presence of π electrons. It can interact with a variety of biomolecules due to its unique properties, which make it compliant with applications in biomedical sectors, like tissue engineering and molecular imaging. Apart from the above applications, rGO has been used in many biological applications, like biosensors, pharmaceuticals, drug delivery, cellular imaging, cancer therapy, diagnostic sensors, and biomarkers [73]. Even in low concentrations, it can cause harm to the environment through bioaccumulation and biomagnification. This widespread use of rGO NPs has triggered the study of its nature and the eco-toxicological effect.
The high surface areas of rGO make it potentially important to help in substrate binding, ROS production, antioxidant deactivation, or ROS scavenging. GFNs react with biomolecules, and in order to use them in the biomedical field, their surface modification is needed. The most common method used for surface modification is covalent modification by the Hummers method to make rGO. Due to the reduction of graphene oxide, there remain a few oxygen-containing groups that enhance cell attachment ability on its surface [83].
Better solubility in water and physiological conditions in comparison to other carbon nanomaterials have shifted all the eyes toward the study of rGO. Tkachev et al. developed a method to prepare rGO. Chemically being alcohol, a reductant was used for the reduction of oxygen-containing functional groups. The TEM study confirmed an ideal hexagonal lattice to provide a large surface area for the binding of different molecules. The available defects were noticed by Raman spectroscopic study. As the C/O ratio plays a great part in structural modulation, the IR spectroscopic and the XPS study showed the reduction of the oxygen-containing groups. The residual oxygen present in water was found to be adsorbed on its surface [84].
Choi et al. prepared rGO by chemical reduction of GO using hydrazine. The quantitative and qualitative characterization was done by XPS. The XPS study demonstrated decreased C/O ratio of rGO [85].
Barra et al. synthesized rGO using caffeic acid by the process of hydrothermal treatment of GO. The XRD study of the crystalline structure showed a significant variance from GO (GO diffraction at 2θ = 10.4°, rGO diffraction at 2θ = 25.7° w.r.t reflection), conforming to the reduction of oxygen-containing functional groups [86]. It showed the radical scavenging property, which could be attributed to the antioxidant activity of rGO [87]. The remaining caffeic acid enhanced the antioxidant property. Wojtoniszak et al. prepared rGO by reducing GO with glucose, which exhibited toxicity to mice fibroblast cells in a concentration-dependent manner [88]. rGO is conjugated with polyethylene glycol (PEG) of hydrophilic nature and can be used for photochemically controlled gene delivery [89]. Osteogenesis is enhanced by rGO-coated hydroxyapatite. It is also used in orthopaedic and dental fillers [90]. Coarse-grained molecular dynamics simulation was used to study the interaction of GFNs, like rGO, with lipid bilayer, suggesting that the rGO particles were localized at the hydrophobic core of the lipid bilayer whereas minimal perturbation occurred when the layer number was small [91]. rGO was utilized in osteogenic stem cells to study [27, 92, 93] chondrogenesis [94], adipogenesis, epithelial genesis [95], myogenesis, cardiomyogenesis [96-98], and neurogenesis. Different methods can be used to improve different sets of properties of rGO for its further potential use (Fig. 6).
Intravenous administration of rGO caused minor transient toxicity in the blood, liver, and kidney of rats. Administration of rGO has shown depletion of endothelial cell adhesion by downregulating the junctional protein and inducing an increment in WBC counts as a protective measure. Graphene-based nanomaterials have been found to be compatible with blood, whereas GO causes stronger platelet aggregation than rGO [69]. It was reported that orally administered rGO caused a short-term decreased locomotor activity and neuromuscular coordinates of the mouse [99]. A few minutes after rGO administration, dispersion of it was observed throughout the brain. Reduced GO changed the blood-brain barrier of rats by decreasing the paracellular tightness of cells [82].
5. SPECIAL ATTRIBUTION OF THE rGO ON ORGANISMS
Reduced GO has some residual oxygen functional groups; thus, it can get soluble in water [99]. An active surface and edges of rGO help it to adhere to cell membranes [70]; as a result, it may block the supply of nutrients to the cells and induce stress. Due to its sharp edge, it can cause membrane damage. Reduced GO is likely to be harmful and could disrupt the membrane integrity by invading it. This process ca trigger apoptosis depending on dose concentration. Due to the less hydrophilic nature of rGO, it was found to adsorb on the cell surface rather than internalizing. The incorporation of rGO in cells is likely to generate ROS and deregulation of antioxidant genes [81].
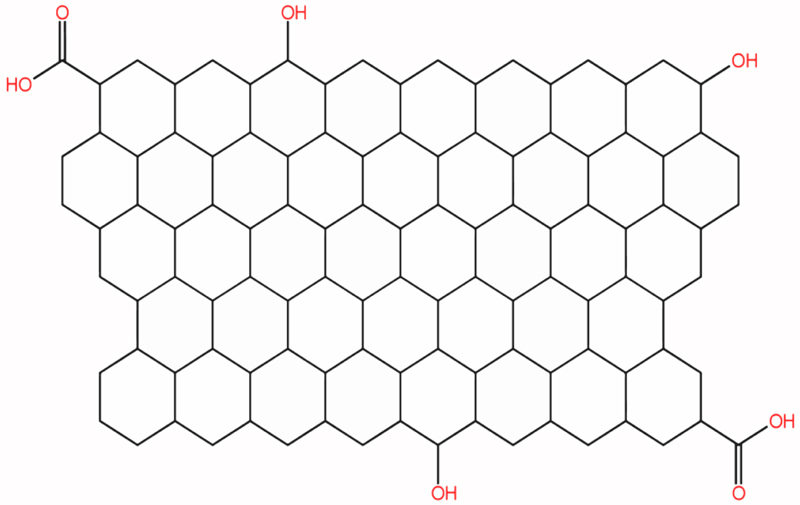
A study on tumor cells has revealed rGO to induce apoptosis to a higher degree than GO. Graphene oxide and rGO both can adhere to cells, but rGO showed a toxic influence on cells, such as degradation of mitochondria, entering capability into cytoplasm and nucleus, alternation in cell morphology, decreased cell adhesion and induction cytotoxicity by increasing the production of intracellular ROS, and triggering cell death [70, 99]. Oral administration of rGO at a high dose accelerated the aging process in mice by reducing the SOD and GPx levels and increasing LPx level [99]. Reduced graphene oxide induced necrosis at high doses [81]. Generally, GO is more toxic than rGO [41]. The study on E. coli showed it to act as a bactericide at high concentrations [48].
To enhance the bioavailability of rGO, it is necessary to inspect its toxicological effects on organisms. This would facilitate the safer implications of it in organisms and the preparation of rGO with less toxicity by adjusting synthesis conditions. A preparation method should be standardized to measure its exact toxicological effects as there are different types of preparation methods to produce rGO with different quantities of oxygen-containing functional groups on its surface [81]. However, the sensitivity of aquatic organisms to rGO is not described. The biological response to NPs can be measured by several parameters, such as the viability of organisms, growth, morphological changes, biochemical changes, and the oxidative status of the organism. Out of all these parameters, oxidative status has been extensively studied to know the toxicological effects of NPs.
ROS elevation, lipid peroxidation, antioxidant imbalance, cellular degradation, and inflammation-causing immunotoxicity, etc., are seen due to the toxic effects of graphene materials in aquatic organisms [74]. Graphene and its derivatives, like rGO, are believed to be biocompatible. rGO-enzyme interaction provides a better picture of the influence on enzyme activity. Oxidation degree plays a great role in this regard, i.e. , the enzymatic conformation and function are quite less with graphene of less oxidation degree [100].
The rGO interacts with nucleic acids, lipids, fatty acids, and proteins, and becomes the cause of oxidative stress by causing dysfunction in macromolecules in different organisms [79]. It causes cytotoxicity with concomitant production of ROS, leading to oxidative stress conditions [72]. rGO caused toxicity in Scenedesmus obliqus by manifesting the down-regulation of PS-II activity with increased oxidative stress, indicating a hike in MDA and ROS levels [101]. A study on nZrO2 combined rGO exposure to the freshwater algae Chlorella pyrenoidosa indicated intracellular oxidative stress and change in membrane integrity augmented by mitochondrial ROS production [102]. The time and concentration-dependent antibacterial activity of rGO on E. coli resulted in oxidative damage to cell membranes, which induced structural modification of bacteria and the destruction of RNA [103]. The human neuronal cell exposure to rGO caused an increase in the activation of caspase-3 and altered the enzymatic activities by producing undue amounts of ROS [104]. Blood platelets on exposure to rGO evoked oxidative stress, causing an imbalance in cell hemostasis and extensive pulmonary thromboembolism [105]. rGO at a nontoxic concentration (up to 50 μg/ml) did not induce any harmful effect; rather, it alleviated Cd toxicity in HepG2 cells of the human liver by suppressing the Cd activity. rGO interaction with lung tissue causes the production of ROS-induced inflammation, apoptosis, and also elevates mitochondrial respiration [106]. Mitochondria, the primary site of ROS production on exposure to rGO, became damaged, leading to metabolic anomalies [107]. Light and air play a pivotal role in stimulating the oxidation of rGO, which in turn causes ROS production in bacteria [108]. Shewanella, a facultative aerobe on exposure to rGO, exhibited stressed condition [109].
Compound | Organism / Cell Lines | Parameters Studied | Results | References |
---|---|---|---|---|
rGO | Mice H9C2 Cells | Lipid peroxidation…………...↑ GSH-PX………………….…...↓ SOD……………………….….↑ |
[73] | |
rGO | HeLa Cell | Lipid peroxidation……… ..…↑ SOD, CAT, GSH-Px………....↓ |
[112] | |
rGO | Liver and kidney of male Wister rat | TBARS Level………..……….No difference SOD……………………….….↑ CAT…………………….........Unaffected |
[69] | |
rGO | Human alveolar cells (A549 cells) |
Did not induce ROS, H2O2 and lipid peroxidation | [113] | |
rGO | HepG2 cells | GSH…………………………..↑ | [111] | |
Co-exposure group (rGO + Pb) | Human alveolar epithelial (A549) cells | GSH, GPx, SOD and CAT……↑ | [113] | |
Thermally reduced GO | Human alveolar epithelial(A549) Cells | GSH…………………………....↑ | [110] |
In toxicological studies of NPs, the major focus has been on oxidative stress. It is widely known that, in a healthy body, the production of ROS and its neutralization by antioxidants is dynamically balanced. Excess increases in ROS production may induce distortion in macromolecules, genotoxicity, mitochondrial dysfunction, and eventually cell death (Table 1).
Reduced graphene oxide has noticeable effects on oxidative stress, cellular activities, and apoptosis activities in comparison to that of GO because of its functional groups, surface charge, and sharp edges, which facilitate its enhanced uptake by the cells [81]. The influence of rGO on the oxidative state of the organism was assessed through the expression of antioxidant enzymes and their activities involved in the detoxification of ROS and xenobiotics. A study has proved that rGO moderately activates oxidative stress and induces some antioxidant activities to restore the balance between oxidants and antioxidants [69]. But it is also said that rGO decreases cell viability and proliferation but at a slower rate than GO in human glioblastoma cells [70].
A study has revealed that rGO induces an increment in ROS levels and reduces antioxidant levels, which are a protective measure against elevated ROS in human lung cells [110]. The same effect was observed in rGO-treated marine mussels. In mussels, rGO evoked immune responses in hemocytes [79]. A study on fish line cells has revealed that GO and rGO lead to cytotoxicity by reducing the mitochondrial membrane potential and inducing a rise in ROS levels [55, 79]. A recent study has shown that a non-cytotoxic rGO concentration effectively alleviates the response of oxidative stress by Cd in HepG2 cells by adsorbing the Cd on rGO sheets [111] Table 1.
It has also been observed that when rGO enters the body, it has harmful effects on organisms as it can integrate or invade the cell membrane and trigger immune and cellular responses by significantly enhancing toxic effects in living systems. Current knowledge about the toxicological implications of rGO is limited, which demands further long-term in vivo investigations in this field. This may help to overcome several challenges before being widely used.
The reduced graphene oxide shows different properties and behaviour compared to graphene oxide. Toxicity of GO, MWCNTs, and RGO was tested on Zebrafish embryos at a concentration of 1, 5, 10, 50 and 100 mg/L for 96 hr; the results revealed the inhibition of hatching zebrafish embryos.
The potential toxic effects of rGO were studied on marine organisms by using in vitro assays with mussel (Mytilus) hemocytes. Cells were exposed to a wide range of concentrations of rGO with PVP to assess cytotoxicity and cell membrane integrity. The results showed invagination and perforation of the plasma membrane, indicating a decrease in cell membrane integrity [79].
6. OVERALL ASPECTS OF THE EFFECTS OF TOXICANTS VERSUS NPS IN ORGANISMS
Toxicants and NPs, including GO and rGO, have specific toxic effects on organisms. Especially the pattern is different in lower organisms in a tissue-specific manner. Previous studies on freshwater snails have shown that the hepatopancreas may be involved in the immobilization and detoxification of metals, as 80% of the total accumulated metals were found in the hepatopancreas and kidney. Due to the bio-concentration activity, aquatic snails are used as bio-indicator for monitoring the presence of toxic heavy metals in aquatic ecosystems. Due to the bioaccumulation of toxicants, edible snails may pose threats to the consumer [114].
Under the stressed condition, a reduction in levels of carbohydrate and protein of P. globosa was observed due to the rapid utilization of energy to combat stress. As there was more protein degradation and less protein synthesis in stressed conditions, the free amino acid concentration was elevated, which can lead to an acid-base imbalance in P. globosa. There was a maximum increase in GST activities as compared to all other antioxidant enzymes like SOD, GPx, and GR. The increased GST activity may be the result of the activation of ROS-induced antioxidant activity [115]. The effect of toxicants on this organism can cause potential damage to the food web. In the hepatopancreas of apple snail, the activity of GST generally increased as a result of Cd exposure [31]. Other freshwater snails, like Lymnaea stagnalis and Lymnaea luteola, are sensitive to copper and cadmium present in water bodies, even at low concentrations.
On exposure to sublethal doses of butachlor, disturbances in protein metabolism were seen in Pila. In response to this, Pila developed a mechanism to reduce metabolic impairment with the help of antioxidants [116]. Nickel, a prominent effluent of industrial waste, causes degradation of protein and subsequent deamination in Pila [117]. On exposure to tannery effluents released into the aquatic environment, the antioxidant activity significantly increased causing cellular damage and protein degradation [115]. Being an important species in the freshwater ecosystem, it is used as a bioindicator to study pollution, and it also has clinical implications.
The whole-body tissues, gonad, and mantle of Pila were subjected to enzyme assays, like SOD, CAT, GR, GSH-GPX, GST, etc., for assessing the toxic impact. Effect of Dithane M-45 on Pila showed alteration in lipase activity level in hepatopancreas and renal organ of Pila at acute and chronic exposure of Dithane M-45. The role of imidacloprid in the total protein content of gill tissue, mantle tissue, foot muscle, hepatopancreas, and male and female gonads of estuarine clam, Katelysia opima, was concluded. It has been found that the protein content of the LC50 group decreased protein in all the cases of the mantel, HP, foot muscle, and male and female gonad as compared to the control.
When snails were exposed to the toxicity of CuSO4, snails released more amount of excreta and showed very little tentacular movement. After 72 hr, all the body parts were retracted into the shell, and no movements were observed [118].
The unique 2D structure and large surface area help rGO to interact with lipid bilayer profoundly. Protein can be adsorbed on the nanomaterial surfaces efficiently, showing toxic responses. Having a larger surface area, rGO possesses larger protein adsorption capacities than other nanomaterials [119]. The interaction of rGO with antioxidants shows it to have a catalytic activity [103]. Conforming to catalytic properties, oxidative damage to anomalies in biochemical pathways is also seen. rGO is less susceptible to oxidative attack than GO by hydrogen peroxide [120]. Catalase eliminates the ROS produced in shrimps due to toxicants and helps in maintaining microbiota homeostasis [121]. In the study of oxidative stress in human alveolar cells (A549), no change was observed in ROS, H2O2, and lipid peroxidation (i.e. , MDA levels) on exposure to rGO; However ROS, H2O2, and MDA levels ameliorated on co-exposure of rGO and Pb [113]. In vivo cardiac toxicity study in mice after administration of rGO revealed the occurrence of lipid peroxidation in myocardial tissues and alteration in myocardial enzymes, exhibiting the oxidative stress condition, and concurrently, the in vitro study on H9C2 cells also showed the same reducing membrane potential of mitochondria [73]. rGO-induced nanotoxicological profile study in rats indicated no such differences in enzymatic activity between the control and treated ones [69].
The study of oxidative stress biomarkers gives us an idea about the effect of toxicants at a molecular level. Animals treated with co-exposure to rGO and β-carotene have shown a reduction in enzymatic antioxidant activity. This reduction might be a result of the increased utilization of the enzyme to dismutate the superoxide ions generated. Due to an elevation in the generation of H2O2, a decline in the GST and catalase activities indicates a compensatory response to the stressed condition. Further, in a long-term response to βC-rGO treatment, the animals exhibited a decline in protein carbonyl contents and lipid peroxides with an elevation in GST levels. In the liver, rGO increases the availability of β-carotene nanocomposite, which results in a huge increase in the antioxidants, like GST activities, to protect the cells from oxidative damage [122].
It can be summarised that NPs in general have specific roles for which they are synthesized [123]. The side effects of NPs in the form of affecting molecular or biochemical pathways are the major lacunae in applying them for wide use. Biochemical pathways, especially the oxidative stress pathways, are specifically regulated in animals. The toxic effects of NPs are molecular pathways-specific, which basically depend on the types of tissue and organs in a species.
CONCLUSION
It is concluded that oxidative stress physiology parameters can be used as effective biomarkers of environmental stressors. Aquatic models can be used for such work. The nanomaterials, including carbon NPs, more specifically the rGO, have been found to modulate the oxidative stress physiology in animals; however, it is tissue and species-specific.
AUTHORS’ CONTRIBUTIONS
BRP contributed to conceptualization, funding acquisition, project administration, collection of resources, supervision, editing, validation, and visualization; FP performed investigation, data curation, writing - review and editing. SGP and AB performed formal analysis, investigation, methodology and software design, and wrote the original draft.
LIST OF ABBREVIATIONS
NPs | = Nanoparticles |
ROS | = Reactive Oxygen Species |
MDA | = Malondialdehyde |
HNE | = Hydroxynonenal |
LPO | = Lipid Peroxidation |
SOD | = Superoxide Dismutase |
GSH | = Reduced glutathione |
AA | = Ascorbic Acid |
G6PD | = Glucose-6-phosphate Dehydrogenase |
CAT | = Catalase |
CONSENT FOR PUBLICATION
Not applicable.
FUNDING
Schemes (number ECR/2016/001984 by SERB, DST, Govt. of India and DST), Govt. of Odisha, India (letter no. 1188/ST, Bhubaneswar, dated 01.03.17, ST- (Bio)-02/2017) to BRP are acknowledged.
CONFLICT OF INTEREST
Dr. Paital is the Associate Editorial Board Member of The Open Biomarkers Journal.
ACKNOWLEDGEMENTS
The authors thank Prof. Pawan Kumar Agrawal, honourable Vice Chancellor of Odisha University of Agriculture and Technology, Prof. CSK Mishra, HoD, Department of Zoology and Director, College of Basic Science and Humanities, Bhubaneswar, for providing laboratory facilities. The help rendered by Dr. Kajari Das during various experiments is acknowledged by the authors. They also express their gratitude to Dr. Shashikant Das for the central instrumentation facility of Odisha University of Agriculture and Technology.